Translation in Neurons
Adam Evans and Jim Hutchins
If you have questions, or want to help in the writing or editing process, please contact hutchins.jim@gmail.com or one of the authors: tessjohnson@mail.weber.edu; calebbevan@mail.weber.edu; jacksonanderson3@mail.weber.edu
Edited by Jim Hutchins on Mar 23 2025
Central Dogma
This 1956 note, made by Francis Crick in preparation for a lecture, is the first known statement of what became the Central Dogma of Molecular Biology. The Central Dogma is simple: information flows from DNA to RNA to protein.
Information in nerve cells is stored in deoxyribonucleic acid (DNA). If unspooled, the DNA in a neuron would be about 3 m long. Because of this, it must be packaged using proteins called histones so it can be packed tightly into each cell.
Genes
DNA is organized into areas which are used to make proteins or regulatory RNAs. These are collectively called genes. There are about 30,000 genes in the human genome.
Each gene has a region that is read before the actual protein coding region. Because DNA and RNA are always read in the 5-prime to 3-prime direction (5’→3′), which is determined by the chemical structure of nucleic acids, the area before the protein coding region is called the 5′ untranslated region (5′-UTR) or the upstream regulatory sequence and the area after the protein coding region is called the 3′-UTR or downstream regulatory sequence. The gene itself consists of regions that will be made into protein (exons) and regions that are not used to make protein (introns).
Regulation of Transcription
While each neuron has 30,000 genes to choose from, each neuron will choose only a small subset of those genes to be expressed — turned into protein. Genes which are specific to being a liver cell or a kidney cell, for example, are permanently turned off in neurons. Also, most neurons turn off the genes needed to support cell division. To permanently turn off a gene, it is wrapped around histones and left in a compact form which does not permit access to the RNA polymerase.
At an even finer level, neurons will only make receptors (for example) when those receptors are needed to replace those which have “aged out” or are otherwise damaged or lost. This is accomplished through transcriptional regulation. A complex loop is formed which binds to transcription factors, complex assemblies of RNA or protein molecules which help the RNA polymerase find its target.
Copying of DNA into RNA
In the process known as transcription, DNA is copied into RNA. In general, DNA is double stranded (i.e. a double helix) while RNA is single-stranded. The enzyme which accomplishes this is RNA polymerase. Bases in the RNA, as it is being made, are matched up to the corresponding bases in DNA, and thymine residues in DNA are replaced by uracil residues in RNA. In that way, each adenine in DNA is matched up to a uracil in RNA; each cytosine is matched up to a guanine; each guanine is matched up to a cytosine; and each thymine in DNA is matched up to an adenine in RNA. This continues until the RNA polymerase encounters the termination site on the DNA double helix.
Splicing
Then after the pre-mRNA (primary transcript) is formed, an organelle called a spliceosome cuts out the RNA which is not part of the protein code (introns) and stitches together the RNA which does code for proteins (exons). A loop of RNA called a lariat is formed, and clipped out. The expressed (i.e. protein coding) regions are tied together to form a string of exons, called messenger RNA (mRNA). It is this mRNA which codes for the protein, as explained in the next chapter. Each mRNA ends in a poly(A) tail: a string of 200 or so adenine residues that will help stabilize the mRNA and ensure that it is translated.
Epigenetics
Permanent chemical alterations in DNA can also change whether genes are more or less likely to be expressed. Because this process is outside of “normal” genetics, it is called epigenetics.
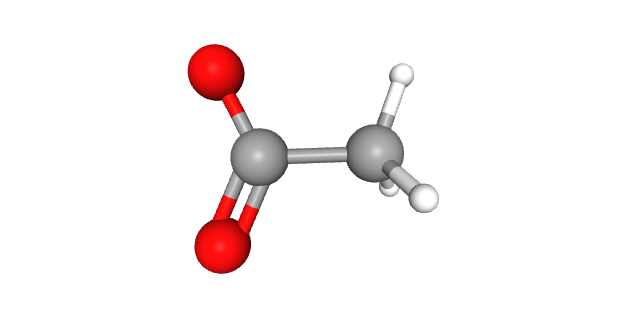
Two of the most common epigenetic modifications of DNA are acetylation and methylation. In acetylation, an acetyl (—CH3COOH) group is added to the DNA, which causes the negatively-charged backbones to repel each other and opens up the configuration of the DNA, making it more accessible to RNA polymerase and therefore more likely to be transcribed.

In methylation, a methyl (—CH3) group is added to cytosine residues on the DNA. This helps compact the DNA structure, making it inaccessible to RNA polymerase and therefore less likely to be transcribed.
or the author: liviaevans@mail.weber.edu
Defining Translation
Once a set of genetic instructions coded in DNA has been transcribed, it exits the nucleus through nuclear pores and into the cytoplasm as mRNA. It is here where the mRNA passes through an organelle called the ribosome. The ribosome effectively holds the mRNA steady and orients it in such a way that it can be translated by tRNA (which, ironically, stands for transfer RNA, not translation RNA). This process, called translation, is how our genetic instructions are converted into proteins.
mRNA: Messenger RNA; carries genetic instructions from DNA out of the nucleus to be translated into proteins
tRNA: Transfer RNA; reads mRNA’s instructions, deposits corresponding amino acids
Codon: A set of 3 nucleotides that code for a particular amino acid or a signal to end protein synthesis; found in mRNA
Anticodon: A set of 3 nucleotides that bind to corresponding codons; found in tRNA
Each tRNA molecule has an anticodon that corresponds to the codons written into the mRNA. When a tRNA molecule finds a match, it deposits the appropriate amino acid, coded for by each codon. Enzymes in the ribosome itself catalyze the formation of covalent bonds (called peptide bonds in this context) between the amino acids, causing them to form a peptide chain. The completed chain, now a polypeptide, can then be folded and assembled into a functional protein following translation.
This is the process of translation that all cells follow in protein synthesis. This includes neurons; however, given the unusual time sensitivity and metabolic demands of even the most mundane tasks of the neuron, natural selection has required them to execute the process of translation more creatively.
Three Steps of Translation:
- Initiation: The start codon at the beginning of the mRNA binds to the ribosome, which orients it so that it is ready to be read; first tRNA molecule binds
- Elongation: The mRNA continues to move through the ribosome, with tRNA molecules depositing the correct amino acids in order to form a peptide chain
- Termination: A stop codon at the end of the mRNA enters the ribosome; tRNA stops binding and the finished polypeptide is released into the ribosome, ready to be folded
Localized Translation
Ordinary animal cells can get by with protein synthesis occurring away from where they are needed, content to wait around for the proteins to be transported after they are built, folded, and packaged at a distant location. Furthermore, their generally compact shapes make protein transfer between subcellular locations rather efficient. Neurons, on the other hand, don’t have such precious time to waste. With long distal projections and highly time-sensitive jobs, neurons utilize localized translation to ensure that the necessary proteins are where they need to be, when they need to be.
Clustered presence of translational machinery outside of neuronal somata was first observed by Steward and Levy in 1982. This seminal finding suggested that neuronal projections (in this case, rat dendrites) were capable of synthesizing proteins on-site, disrupting the long-standing assumption that they were dependent upon highly targeted transport of proteins already synthesized in the soma. While initially controversial, the concept of localized translation is now widely accepted by neuroscientists.
Functional Importance of Localized Translation in Neurons
Imagine you need to make sandwiches in a commercial kitchen in New York, which is about the size of a warehouse (40 m or 120 feet across). You want to get those sandwiches to Los Angeles, across the North American continent, a distance of 4000 km (2450 miles). That’s pretty difficult, but now imagine everything you send across the continent has to travel through a large sewer pipe (0.4 m = 1.2 foot diameter).
That’s the problem for neurons. Shrink the example above by a factor of 4,000,000, and you have a cell body with diameter 10 μm, axon length 1 m (a very tall person has sensory axons this length) and axon diameter 0.1 μm. Organelles and most proteins must be made in the cell body and shipped via microtubule “rails” with kinesin “engines”. Amazingly, these engines travel at 0.4 m per day, so the trip can be as short as 5 days from cell body to axon terminal. Still, this represents a significant impediment to a dynamic body that may be encountering changing circumstances. For example, if we are out of the neurotransmitter substance P (a pain signal), then it will take almost a week to resupply. Substance P and other peptide neurotransmitters are made in the rough endoplasmic reticulum of the cell body, packaged into vesicles, and transported to the axon terminal.
This scenario represents the dilemma of neurons, who can’t always afford the gamble that comes with dependence on timely imports of pre-assembled proteins from the soma. In our specific example, we’ve represented the human unipolar sensory neuron.
Apart from these time constraints, trafficking proteins across long distances can pose a risk to the integrity of the proteins themselves, even at low quantities. Mitigating this risk proves to be rather costly to the cell, especially through crowded cytoplasmic environments (pictured left). Ensuring safe transport of proteins is an enormous biological task for all cells.
During transport, proteins must be escorted by chaperone proteins to prevent misfolding, repair lapses in correct folding, and prevent and break up protein aggregates. These dangers are especially present when travelling through crowded through these cytoplasmic environments. Furthermore, they must be packaged into sophisticated protective vesicles preceding transport, which are very expensive for the cell to synthesize and maintain.
Chaperone proteins: Proteins that help fold proteins into the correct shape, as well as maintain it
Protein aggregate: A large mass of proteins, usually misfolded proteins
Vesicle: A sac that transports substances within or out of a cell, consisting of a membrane bilayer with imbedded proteins
Between the ATP used in translation and the cost of acquiring essential amino acids, synthesizing and folding proteins is already biologically costly enough. The time and energy needed to transport these precious goods, combined with the risk of damage (and the enormous cost in preventing it) when doing so, it’s no wonder that evolution has incentivized us to avoid it altogether.
With mind to our “commercial kitchen” analogy, consider the advantages of making sandwiches on the West Coast, rather than 4000 m away. It reduces the supply chain issues considerably.
Locally translating a portion of their proteins, rather than solely relying on long-distance transport, saves neurons valuable energy and resources–especially with their distinctively high metabolic requirements in mind. It also saves them precious time in handling their urgent demands. (Because of this metabolic demand, in diseases of metabolism such as diabetes, the longest axons are the first to malfunction.)
Localized Translation in Dendrites
Dendrites have been shown to be capable of translation. As many as 90% of synapses are capable of postsynaptic translation; among the majority of these dendrites, localized translation occurs even without exogenous stimulation. Their role in maintaining synaptic function is so important that many proteins are produced primarily (or even exclusively) within dendrites.
The postsynaptic density (PSD) is a massive protein complex present in the postsynaptic chamber of excitatory synapses. As the name would suggest, it is, in fact, quite dense! Between the receptors, cytoskeletal elements, scaffold proteins, and signaling enzymes needed to maintain function at the postsynaptic terminal, the slender tips of dendritic spines are often packed wall-to-wall with proteins. This can be observed in the figure to the right; the postsynaptic terminals are notated with an asterisk, and the arrow points toward the PSD itself, a dark region dense with protein. Additionally, the postsynaptic cells themselves are visibly darker due to their elevated protein content.
While we have documented a major portion of the individual proteins that comprise the PSD, there is much left to learn, particularly on the molecular level, where specialized 3D structure and function remains unclear. The same is true, to an even greater extent, for the protein complexes of inhibitory postsynaptic terminals. What we do know is that they are essential to neural development and function, with deficiencies in the protein complexes of both inhibitory and excitatory synapses correlating with many neurodevelopmental, neurofunctional, and neurodegenerative disorders.
Possibly because of their demanding protein requirements, nearly all localized translation occurs within the dendrites, where the phenomenon was first observed. In fact, the majority is so vast that the existence of axonal translation past development remains a topic of controversy in the scientific community.
Localized Translation in Axons
During development of the nervous system, localized translation within axons is common and essential in virtually every aspect of axonal development, particularly in the upkeep and function of axonal growth cones. Ribosomes are found in growth cones, and when the growth cone matures into a synapse, those ribosomes disappear. This provides easy access to proteins that promote synaptogenesis. However, after pruning has occurred and synaptogenesis has occurred, translational machinery in axons quickly and dramatically decreases, often to nearly undetectable levels.
Growth cones: The tips of developing axons that guide the growth of neurons to their targets.
Electron microscopic observations from the 1950s and 60s have largely shown a lack of any ribosomes within the mature axon whatsoever. Despite random sightings of mature axonal ribosomes within the same time period, neuroscientists have operated under the presumption that mature axons lacked the capacity to locally synthesize proteins for over 50 years. More recent publications have disrupted this canon, suggesting that mature axons in both invertebrate and vertebrate species are indeed capable of local protein synthesis. In fact, it has been observed in mice that over 75% of presynaptic terminals contain translational machinery. Furthermore, 37% are actively translating proteins at any given moment (lagging behind postsynaptic terminals, of which 61% are actively translating at a given moment).
Within the peripheral nervous system, ribosomes are frequently found within the axons of mature neurons that have sustained injury. It appears that these ribosomes are donated by Schwann cells and aid in and restoring axonal length and connectivity. Within the central nervous system, it has been proposed that localized translation within CNS axons is crucial to long-term maintenance and function.
While the subject of localized translation within mature axons remains a topic of controversy, contributions to the literature over the past two decades have reflected a growing curiosity in the subject. Despite the publication of increasingly compelling evidence that mature axons are, in fact, capable of local protein synthesis, the number of answers we’ve uncovered doesn’t hold a candle to the number of questions and doubts we still face (particularly in respect to the CNS). All things considered, recent findings cautiously point us toward localized translation in the mature axon as, if nothing else, a strong possibility.
mRNA Transport Mechanisms
In order to be translated locally in the dendrites or axon, it must first be transported there. Like in any other cell, mRNA first emerges from nuclear pores and into the surrounding cytoplasm. When considering how far these thousands of tiny mRNAs must travel to reach their final localized destinations, it’s easy to wonder if this is really all that much more efficient. Compared to the extravagant metabolic costs of protein transport, however, shipping mRNA across the cell is relatively cheap, and absurdly fast.
Soon after they reach the cytoplasm, mRNA is bound by a series of RNA binding proteins (RBPs) that tag them for transport to their final destination (e.g., a certain dendrite). This forms an messenger ribonucleoprotein (mRNP) complex. Like many things in molecular biology, where there is one mRNP complex, there are many. These complexes are chemically attracted to one another, and self-package into large, easily-transportable granules.
RBPs: RNA-binding proteins; bind to RNA to regulate gene expression by controlling when and where, and whether or not, mRNA is translated.
mRNP complex: A single mRNA molecule bound by several RBPs; the basic functional unit regulating mRNA processing, export, localization, translation, and degradation
Granule: A large, transportable aggregate of mRNP complexes
Like in the transport of protein-carrying vesicles, the granule is carried to its destination by motor proteins, including kinesin (pictured) and dynein, along longitudinal tracks of structural proteins. These proteins and their role in retrograde and anterograde transport are discussed in greater detail in a later chapter.
Regulated Translation and Synaptic Plasticity
While some mRNAs are translated immediately upon arrival, many are held in a “repressed” state until needed. Apart from the sheer purpose of avoiding wasted effort, this process has remarkable utility. The strict regulation of when proteins are synthesized allows the brain to engage in stimulus-dependent protein synthesis, meaning that the translation of mRNAs is only triggered by certain events. This effectively allows us to “customize” our synapses based on their patterns of use.
It was first demonstrated over 50 years ago that the formation of memories in mammals requires protein synthesis, and hypothesized a further 25 years earlier. This is true for both LTP and LTD, both crucial mechanisms in long-term memory consolidation. Both of these processes are marked by stimulus-dependent synthesis, as well as the use of unique proteins. These conditions imply that the tight regulation of mRNA translation is required.
We already know that many RBPs are involved in tagging mRNA for transport, but their jobs do not end there. Certain RBPs are also involved in inhibiting translation. The conditions under which they repress mRNA translation, as well as the manners in which they do so, are as diverse as the proteins themselves.
For example, zip code binding protein (ZBP1) prevents mRNA from being prematurely translated before it’s localized to its final destination. Others, such as fragile X mental retardation protein (FMRP), stall the ribosomes after translation has already begun. They bind to mRNAs in a way that mechanically “blocks” the ribosome from continuing along the mRNA during the elongation phase. As the name suggests, mutations in FMRP are associated with the intellectual disability and synaptic dysfunction seen in those with the genetic disorder Fragile X syndrome.
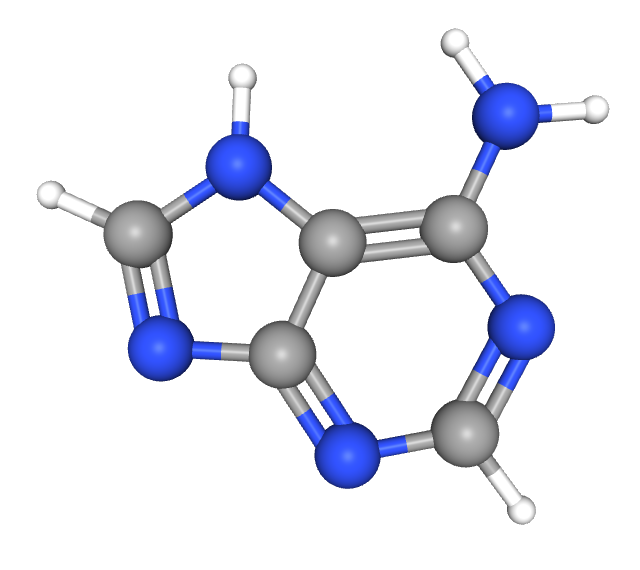
Poly(A) tail: A string of about 250 adenine (A) nucleotides attached to the 3′ end of mRNA; provides stability and regulates translation by increasing affinity
Repression of mRNA translation is also impacted by the structure of the mRNA itself. This plays out in two key ways, the first of which involves poly(A) tail length. Pictured below, this humble string of adenine plays a critical role in both mRNA stability and regulation of translation. A long poly(A) tail promotes protein synthesis by recruiting poly(A)-binding proteins (PABPs), which help initiate translation by recruiting translation initiation factors, ultimately increasing binding affinity with ribosomes. In neurons, mRNAs are often transported with shortened poly(A) tails, rendering them translationally inactive.
One of the key regulators of poly(A) tail length in neurons is Cytoplasmic Polyadenylation Element-Binding protein (CPEB), which binds to specific sequences in the 3′ untranslated region (3′ UTR). Once bound, they maintain their target mRNA’s translationally repressed state. This is achieved through the prevention of polyadenylation, or the rebuilding of a “clipped” poly(A) tail, keeping them translationally dormant. However, when synaptic activity occurs, signaling pathways (such as those involving CaMKII [LINK TO CHAPTER HERE]) can activate CPEB, prompting it to extend the poly(A) tail, allowing translation to proceed. This mechanism is particularly important for the stimulus-dependent protein synthesis at synapses required for LTD and LTP.
On the other end of the mRNA sits a 5′ cap, a modified guanine (G) nucleotide at the 5′ end of mRNA. Neuronal mRNA is regulated, in part, by cap-binding proteins, which can either promote or inhibit translation. Regulation using cap-binding proteins is especially tight, seeing as the 5′ cap is the initial site where translation begins. The 5′ cap attracts cap-binding proteins, which are necessary to catalyze protein synthesis.
Eukaryotic Initiation Factor 4E (eIF4E) is the primary cap-binding protein that recruits the ribosome to the mRNA. In neurons, eIF4E activity is controlled by eIF4E-binding proteins (4E-BPs). Under normal conditions, 4E-BPs stubbornly bind to eIF4E, preventing it from initiating translation.
However, when synaptic stimulation occurs, phosphorylation of 4E-BPs causes them to lose their affinity for eIF4E. The 4E-BP releases itself from the eIF4E, allowing translation to proceed. Similar to CPEB, 4E-BPs ensure that target mRNAs are only translated in response to the relevant stimuli.
Certain 4E-BPs in humans, such as 4E-T, have been observed to play the dual role of inhibiting translation through deadenylation and through preserving the target mRNA’s 5′ cap. 4E-T is found extensively in human neurons, suppressing synthesis of certain proteins until the appropriate stimulus is received. The unique manner in which 4E-T functions allows mRNA to be stored in a state of deep dormancy while avoiding the all-too-inevitable: mRNA decay.
Mechanisms for Preventing mRNA Decay
We’ve established that the journey of an mRNA to a distal projection can be extremely long. When combined with the time that it spends at its final destination waiting to be translated, an mRNA molecule may be “sitting out” for quite a long time. Just like any other biological material, mRNA has an expiration date, and is likely to degrade if no extra care is put into preserving it.
Unlike most cell types, neurons require long-lived mRNAs to support their uniquely urgent and high-volume protein demands. In order to ensure that localized transcripts remain available for translation, especially after they’ve gone through the trouble of transporting them, neurons implement highly specialized routines to stabilize and prolong the lifespan of mRNA.
Neurons loved RBPs so much, they got one in every color. In addition to regulating mRNA transport and translation, RBPs are also utilized in mRNA stabilization. They associate with elements in the 3′ UTR of target transcripts to protect them from degradation. HuD, for example, can approximately double the half-life of axonal poly(A)+ mRNA it associates with. The presence of HuD and other RBPs ensures that neuronal mRNAs persist until they are needed for translation, particularly in response to synaptic activity. So chic.
[**NOTE: The article I just linked is CC, so we could use their figures if we really wanted to. I just didn’t find any very effective, but we should still take a look]
The structure of the mRNA itself plays an crucial role in regulating degradation, just as it does in translation. There are two major pathways by which mRNA decay is often initiated, both directly tied to the end structures of mRNA. This necessitates that RBPs involved in mRNA preservation largely focus their efforts toward protecting these end structures.
Deadenylation-dependent decay occurs when the poly(A) tail of an mRNA is removed, leaving transcripts unstable. This process also leaves the 3′ end open for uridylation, which marks mRNAs for destruction by recruiting 3′ to 5′ exonuclease.
While this process is important for processes like dynamic gene silencing, failing to control when and where uridylation occurs can lead to the lifespan of mRNAs being cut short. To ensure that the poly(A) tail is protected, neurons recruit PABP, which both inhibits uridylation and mechanically prevents direct exonuclease access. While this protein is often used to catalyze translation, it can also preserve dormant, short-tailed mRNA, able to stably bind to poly(A) tails as short as 12 nucleotides long.
Decapping-dependent decay, as the name more directly suggests, occurs when the 5′ cap is removed in response to deadenylation at the 3′ end. The 5′ cap is strictly required for translation to begin, meaning that removal renders the mRNA completely inactive. Decapping makes mRNAs extremely susceptible to rapid destruction by 5′ to 3′ exonuclease.
While promoting overall transcript stability from the 3′ end can indirectly inhibit decapping at the 5′ end, some proteins are directly involved in protecting the 5′ cap. Recall 4E-T’s unique ability to suspend mRNA in dormancy for long periods of time. The key to this is in the structure—4E-T mechanically blocks the catalyzation of 5′ decapping by Dcp1-Dcp2. By this process, deadenylated mRNAs are allowed to be stored, rather than destroyed.
[Good source, still need to synthesize most compelling info. CC-BY license, so we can use their figures if we want: https://doi.org/10.1101/2025.03.21.644491]
UNDER CONSTRUCTION
- m6 RNA mods