Energy Metabolism in Neurons
Jackson T. Anderson; Paulina Michór; Tess Johnson; and Caleb Bevan
This is a near-final draft which is in the process of being edited. If you have questions, or want to help in the writing or editing process, please contact hutchins.jim@gmail.com or one of the authors: jacksonanderson3@mail.weber.edu tessjohnson@mail.weber.edu; calebbevan@mail.weber.edu.
Primary Energy Sources
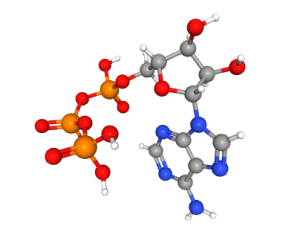
The human brain accounts for approximately 2% of total body mass, yet it is the most energy-intensive organ, consuming nearly 20% of the body’s overall energy expenditure. Remarkably, about 75% of the brain’s energy is dedicated to neurotransmission and essential cellular processes. Adenosine triphosphate (ATP), a nucleoside triphosphate abundant in neurons, is often called the “energy currency” of cells and plays a crucial role in a wide range of cellular functions.
In synaptic transmission, a large amount of ATP is essential for synthesizing and loading neurotransmitters into vesicles and preparing these vesicles for fusion and subsequent recycling. It also plays a vital role in maintaining energy levels and is crucial for preserving the cell’s resting ion gradient, thereby preventing excitotoxicity caused by excess glutamate. Excitotoxicity can result in calcium influx and oxidative stress, ultimately leading to neuronal cell death. Furthermore, ATP powers motor proteins such as kinesin and dynein, which facilitate the transport of cellular materials along long axons. In summary, ATP is a fundamental direct and indirect component of numerous neuronal processes. This significance prompts several questions regarding the creation and regulation of ATP, which we will examine in further detail.
Glucose Transport into Neurons
Glucose is transported into cells through sodium-independent glucose transport (GLUT) or sodium-dependent glucose transport (SGLT) which is also called sodium-linked glucose transport. Each of these processes is carried out by a family of proteins which carry the GLUT or SGLT name followed by a number.
Unlike other tissues, glucose transport in the brain operates independently of insulin. The way neuronal cells absorb energy substrates varies depending on the specific type and distribution of transporters unique to each cell type.
There are three steps in glucose transport into neurons. In the first two steps, glucose makes its way across the blood-brain barrier (BBB). First, glucose must be transported through the endothelial cell lining of the capillary by GLUT1. It then is taken up by GLUT1 on the surface of astrocytes. In the third step, astrocytes pass glucose with GLUT1 from the intracellular to the extracellular space near neurons. Because gluocse concentrations in the brain are low, the brain depends on a high-affinity glucose transporter (GLUT3) to capture all glucose made available to them.
Key Metabolic Pathways
Glucose Metabolism
In summary, ATP is created through several cellular processes: glycolysis, the tricarboxylic acid cycle, and oxidative phosphorylation. All of these entail the oxidization of glucose, which results in the production of ATP, which occurs through a combination of glycolysis and the citric acid cycle. Glucose is the primary energy source for the adult brain; in other words, our brains have a sweet tooth. The equation for glucose oxidation is as follows: C6H12O6 + 6 02 ⇒ 6 CO2 + 6 H20. This equation represents the complete oxidation of glucose in which six oxygen molecules are required to oxidize one glucose molecule, resulting in a molar ratio of 6:1.
Nearly all glucose in the brain is oxidized at its resting rate, and during periods of brain activation, glucose utilization increases significantly more than oxygen consumption. The rise in cellular activity associated with specific brain functions heightens the local demand for ATP, stimulating the flux of metabolic pathways, ATP production, and blood flow to the activated tissues. Conversely, decreased activity leads to downregulation of glucose and oxygen consumption and reduced blood flow.
Glycolysis
Glycolysis, the first stage of cellular respiration consists of 10 enzymatic reactions that extract energy by converting a glucose molecule into two three-carbon molecules known as pyruvate. The initial step involves the irreversible phosphorylation of glucose facilitated by hexokinase. This process occurs in the cytoplasm and results in a net gain of two ATP molecules for each glucose molecule metabolized, even though two ATP molecules are initially consumed. The reactions catalyzed by phosphoglycerate kinase and pyruvate kinase are responsible for the production of ATP in this pathway.
Glycolytic enzymes that are membrane-bound, such as hexokinase and phosphoglycerate kinase, operate alongside ion pumps (see chapter on Pumps), including Na+/K+-ATPase, H+-ATPase, and Ca2+-ATPase, all of which are directly powered by ATP. This localized ATP production through the glycolytic pathway is crucial for fulfilling high energy demands rather than relying solely on ATP generated by mitochondria elsewhere in the cell. While glycolysis yields only a tiny amount of energy compared to other metabolic pathways, it is a vital connection to various metabolic processes. For instance, pyruvate, an end product of glycolysis, acts as the starting point for the tricarboxylic acid (TCA) cycle.
Malate-Aspartate Shuttle
During glycolysis, one glucose molecule produces 2 molecules of nicotinamide adenine dinucleotide (NADH) from NAD+ molecules. This conversion occurs through a reduction reaction, meaning that NAD+ accepted electrons, and become NADH. The electrons carried by NADH will then be used in the electron transport chain to produce more ATP through oxidative phosphorylation. However, the inner mitochondrial membrane is impermeable to NADH. To achieve the transport of these electrons from the cytosol into the mitochondria, a metabolic pathway called the malate-aspartate shuttle is utilized.
First, the cytosolic enzyme malate dehydrogenase (MDH1) converts oxaloacetate to malate using NADH as cofactor. Malate can then be transported into the intermembrane space and into the mitochondrial matrix via a malate-alpha-ketoglutarate transporter. Once in the mitochondrial matrix, malate is oxidized back into oxaloacetate, reducing NAD+ to NADH, via the mitochondrial enzyme malate dehydrogenase. The NADH that is now inside the mitochondria and can enter the electron transport chain (starting with complex I).
The oxaloacetate produced during this process cannot cross the inner mitochondrial membrane and therefore needs converting into aspartate in a transamination reaction via an enzyme called aspartate aminotransferase (AST). Aspartate can cross the mitochondrial membranes back into cytosol through a glutamate/aspartate antiporter. The glutamate that has just entered the mitochondrial matrix gives its amino group to oxaloacetate to form aspartate and becomes 2-oxoglutarate itself (also known as α-ketoglutarate). The 2-oxoglutarate can be transported via malate-α-ketoglutarate transporter into cytoplasm, where it gets converted to glutamate in a reductive amination reaction. The aspartate that entered the cytoplasm is deaminated to form oxaloacetate, which is available to start the malate-aspartate shuttle cycle again.
The Tricarboxylic Acid (TCA) Cycle (Citric Acid Cycle, Krebs Cycle)
The tricarboxylic acid (TCA) cycle, also known as the citric acid cycle or Krebs cycle, constitutes the second stage of cellular respiration and occurs within the mitochondrial matrix. This localization is a primary reason for the mitochondria’s designation as the “powerhouse of the cell.” The principal function of the TCA cycle is to generate high-energy electron carriers, specifically nicotinamide adenine dinucleotide (NADH) and flavin adenine dinucleotide (FADH₂), through a series of enzymatic reactions. These electron carriers subsequently participate in the electron transport chain to facilitate the production of adenosine triphosphate (ATP), the principal energy currency of the cell. Furthermore, the TCA cycle produces various metabolic intermediates, such as α-ketoglutarate and oxaloacetate, which serve as precursors for vital biosynthetic processes. For instance, α-ketoglutarate is a critical precursor for glutamate, the predominant excitatory neurotransmitter in the brain, and its derivative, γ-aminobutyric acid (GABA), is an important inhibitory neurotransmitter.
The TCA cycle is initiated by the condensation of acetyl-CoA with oxaloacetate to yield citrate, a reaction catalyzed by citrate synthase. Through subsequent enzymatic transformations, citrate is converted sequentially into isocitrate, α-ketoglutarate, succinyl-CoA, succinate, fumarate, malate, and ultimately regenerates oxaloacetate. During this process, two molecules of carbon dioxide are released, alongside the production of three molecules of NADH, one molecule of FADH₂, and one molecule of guanosine triphosphate (GTP) or ATP per cycle turn.
Regulation of the TCA cycle within neurons is critical to align metabolic activity with energy demands. Key regulatory enzymes, including pyruvate dehydrogenase (PDH), isocitrate dehydrogenase, and α-ketoglutarate dehydrogenase, are pivotal control points in this metabolic pathway. PDH, for example, controls the entry of acetyl-CoA into the cycle and is subject to inhibition by elevated levels of ATP, NADH, or acetyl-CoA, thereby signaling sufficient energy availability. Conversely, calcium ions, often released during synaptic activity, activate various TCA cycle enzymes, establishing a link between energy production and neuronal firing.
Biosynthetic Pathways: Neurotransmitter Synthesis & Repair
The brain utilizes many different neurotransmitters for various functions. These need to be synthesized in the soma (neuropeptides) or they can be synthesized in the axon terminal (small molecule neurotransmitters). The synthesis can happen either from the specific precursors or from cellular metabolites, depending on the neurotransmitter. Neurotransmitters are then packaged into synaptic vesicles and, in the case of neuropeptides, transported to the axon terminal, where they can be used for neurotransmission. Following the neurotransmitter release into the synapse, the neurotransmitter is removed from the synaptic cleft back into the nerve terminal (reuptake). Reuptake allows for termination of the neurotransmitter signal, as well as for energy-efficient recycling of the neurotransmitters.
Differences in neurotransmitter synthesis and repair exist depending on whether the neurotransmitter is a small molecule (amino acids, monoamines), a peptide (neuropeptides, opioids), or other.
Oxidative Phosphorylation
The final step in energy production is cellular respiration, the primary source of ATP in neurons. Oxidative phosphorylation can be divided into two key components: the electron transport chain (ETC) and chemiosmosis. As previously stated, the primary function of the TCA cycle is to generate NADH and FADH₂, which are subsequently transferred through protein complexes in the electron transport chain (ETC) located in the inner mitochondrial membrane. As electrons traverse Complexes I, III, and IV, energy is released, which is then utilized to pump protons into the intermembrane space, establishing an electrochemical gradient. Oxygen acts as the final electron acceptor in Complex IV, resulting in the formation of water. This proton gradient drives ATP synthase, synthesizing ATP as protons flow back into the mitochondrial matrix.
The electron transport chain produces reactive oxygen species (ROS) as a byproduct. These ROS are mainly produced as a result of a premature leak of electrons from complexes I, II and III. A single electron has an ability to reduce an oxygen molecule to superoxide (O2•−). These highly reactive ROS can damage mitochondrial proteins, membranes and DNA, which could lead to oxidative stress and impairment in mitochondrial function.
To counteract these harmful effects of oxidative stress, pathways that upregulate antioxidant expression such as heat shock protein 70 (HSP70) are activated. HSP70 can act through a variety of ways, one of which is binding to and stabilising proteins, to protect them against denaturing action of ROS. HSP70 can also help with degradation of already oxidized proteins.
Pentose Phosphate Shunt (PPS)
The pentose phosphate shunt (PPS) is a metabolic pathway parallel to glycolysis, taking place in the cytoplasm. During the PPS, which contains oxidative and non-oxidative phases, glucose-6-phosphate (produced during glycolysis or through other mechanisms) generates pentoses (sugars) and carbon dioxide. Pentose sugars (ribose and deoxyribose) are an integral part of ribonucleic acid (RNA) and deoxyribonucleic acid (DNA), respectively. The PPS is therefore a crucial metabolic pathway for the production of DNA and RNA.
The PPS also converts nicotinamide adenine dinucleotide (NAD+) to nicotinamide adenine dinucleotide phosphate (NADPH), which similarly to NADH can be used as electron donor. NAD+ carries two electrons and one proton into other metabolic pathways.
The PPS also kicks into gear when neurons are under oxidative stress. Mitochondrial activity necessarily produces free radical oxygen species; nitric oxide (NO) is used as a signaling molecule and also can produce free radicals. These electrons can be transferred to NADPH, but that makes this key metabolite unavailable for energy production and reduces the normally huge energy production needed to maintain neurons. Over time, this can cause damage which may lead to disease.
Alternative Energy Sources
The Astrocyte-Neuron Lactate Shuttle Hypothesis
Neurons do not just rely on glucose as an energy source. One alternate energy source is lactate (the ionic form of lactic acid), which is a by-product of glycolysis. The astrocyte-neuron lactate shuttle hypothesis was proposed in the mid-1990s. According to this hypothesis, when neural activity increases, astrocytes increase the rate of glucose uptake. This means that more glucose is burned anaerobically (i.e. without sufficient oxygen to enter mitochondria) and lactate is produced. The lactate then is transported into neurons via the monocarboxylate transporter 2 (MCT2) and converted to pyruvate in neurons by the enzyme lactate dehydrogenase 1 (LDH1) using NAD+ as a cofactor. The pyruvate then enters the mitochondria and is converted to ATP by the citric acid cycle and oxidative phosphorylation.
Ketone Bodies
Ketones are another alternative source of energy for neurons. Ketones are formed when blood glucose levels are low, such as in the fasting state. A ketogenic or low-carbohydrate diet is used to deliberately increase ketone levels in the bloodstream. Ketone bodies are formed from free fatty acids predominantly in the liver but can also be produced by astrocytes in the brain. Ketone bodies can then enter neurons via MCT2, where they get converted by a series of reactions into acetyl coenzyme A (acetyl-CoA), which then enters the citric acid cycle in neurons.
Alternatively, when fatty acids enter the astrocytes from capillaries through fatty acid transport proteins (FATPs), they can be β-oxidized into β-hydroxybutyrate (BHB), and then ketolysed into acetyl-CoA, which enters the citric acid cycle and leads to ATP production within astrocytes.
Glycogen Storage
The brain does not contain a large storage of glycogen, especially when compared to the liver or skeletal muscle. However, glycogen is still found in the brain, predominantly in astrocytes, which can rapidly metabolize it to lactate to provide energy to neurons. Neurons contain lower concentrations of glycogen, compared to astrocytes, which can be metabolized in hypoxia. More recently, glycogen in the brain has been investigated beyond these metabolic functions and has been found to be involved in memory and learning, as well as brain homeostasis.
Glycogen synthesis starts with glucose transport into the brain from the blood via blood brain barrier and via the GLUT family described above. Glycogen is then formed from glucose-6-phosphate by an enzyme called glycogen synthase, and by the branching enzyme, which overall makes the glycogen a compact glucose polymer for a readily-available glucose storage.
Astrocytic Lactate
The astrocytic metabolism of glycogen to lactate can be explained by the astrocyte-neuron lactate shuttle (ANLS). In response to an enhanced neuronal activity, astrocytes can metabolize both glucose and glycogen to produce lactate, which is released to the extracellular space via monocarboxylate transporters (MCT), specifically via MCT1 and MCT4. Neurons are able to take up lactate via MCT2 and use it as an additional source of energy (by conversion to pyruvate).
Specializations of Energy Metabolism in Other Glial Cells
Microglia
Microglia are the resident immune cells of the brain with a main function to destroy invading pathogens, and many other functions. Under physiological conditions, traditionally considered “resting”, microglia produce ATP mainly through oxidative phosphorylation. However, when “activated” in response to proinflammatory stimuli, microglia switch to ATP production through glycolysis.
Oligodendrocytes
Oligodendrocytes are a type of glial cells that are the myelinating cells of the central nervous system. They sheath the axons from the extracellular space, so that action potential generation happens through saltatory conduction at the unmyelinated nodes (nodes of Ranvier). This process is energy-efficient and leads to faster signal conduction. Oligodendrocytes utilize glucose and lactate for glycolysis and oxidative phosphorylation to generate ATP. Oligodendrocytes also play an important role in providing energy sources to myelinated axons. Oligodendrocytes metabolize glycose to lactate and release it via MCT1, which can then be taken up by axons via MCT2. They can also release glucose via GLUT1, so that axons take it up via GLUT3. The unmyelinated nodes are metabolically supported by astrocytes instead.
Alternatively, under low glucose conditions, oligodendrocytes rely on fatty acid β-oxidation in mitochondria and peroxisomes for energy production.